What affects the depreciation grid storage batteries, especially in the range betweek 30kWh to 1MWh?
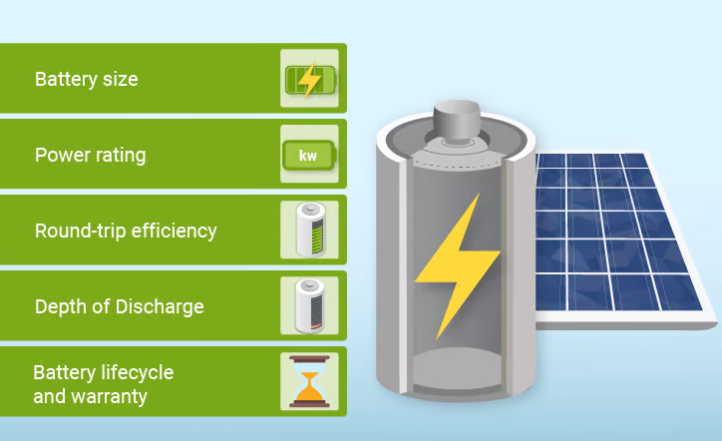
Battery depreciation, also known as battery degradation, is a process that occurs over time and affects the capacity and performance of batteries, including those in the range of 30 kW to 1 MW. Several factors can contribute to battery depreciation, including age, the number of charge-discharge cycles, temperature, and usage patterns. Let’s explore these factors in more detail:
- Age: Over time, even if a battery is not heavily used, it will naturally degrade. Chemical reactions within the battery lead to the breakdown of its components, reducing its capacity and overall performance.
- Number of Cycles: One of the primary factors affecting battery depreciation is the number of charge-discharge cycles it undergoes. Each cycle typically involves charging the battery to its full capacity and then discharging it. The more cycles a battery goes through, the more it will degrade. Batteries are often rated for a certain number of cycles before their capacity significantly drops.
- Depth of Discharge (DoD): Deeper discharges, where the battery is drained to a lower state of charge before recharging, can accelerate degradation. Shallower discharges, where the battery is only partially depleted before recharging, tend to be less stressful on the battery and can prolong its lifespan.
- Temperature: Temperature has a significant impact on battery degradation. High temperatures can accelerate chemical reactions within the battery, leading to faster degradation. Conversely, lower temperatures can slow down these reactions, potentially extending the battery’s life. Proper thermal management is crucial for maintaining battery health.
- Charging and Discharging Rates: Rapid charging or discharging at high rates can generate heat and stress the battery, leading to faster degradation. Slower, more controlled charge and discharge rates are generally less damaging to the battery.
- Chemistry and Design: Different battery chemistries (e.g., lithium-ion, lead-acid) have varying rates of degradation. Newer battery designs and chemistries may offer improved durability and longer lifespans compared to older technologies.
- Maintenance and Management: Proper maintenance and management of battery systems can help mitigate degradation. This includes ensuring balanced charging and discharging of cells in multi-cell systems and using battery management systems (BMS) to monitor and control battery performance.
- Calendar Aging: Even if a battery is not used much, it will still degrade over time due to calendar aging. This is why some manufacturers recommend replacing batteries after a certain number of years, regardless of usage.
- Manufacturing Quality: The quality of manufacturing and the materials used in the battery can also affect its lifespan. High-quality batteries tend to degrade more slowly than lower-quality ones.
- Environmental Conditions: Environmental factors such as humidity, exposure to contaminants, and vibrations can also impact battery longevity.
It’s important to note that the rate of battery depreciation can vary significantly depending on these factors and the specific battery chemistry and design. Manufacturers often provide data on expected battery lifespan and degradation rates for their products, which can help users estimate how long a battery is likely to last under specific conditions.
Regular monitoring, maintenance, and following manufacturer guidelines for battery usage and maintenance are essential for maximizing the lifespan and performance of batteries in the 30 kW to 1 MW range or any other size.
What is the typical depreciation curve agains one of the factors?
The typical depreciation curve of a battery against one of the factors, such as the number of charge-discharge cycles, follows a nonlinear pattern. Here’s a simplified representation of how battery capacity tends to degrade over time due to cycling:
- Initial High Capacity: When a battery is new, it typically has a high capacity and performs at its best. It can hold a substantial charge and deliver its rated power.
- Early Rapid Decline: As the battery undergoes its first few cycles, there may be a noticeable but relatively slow decline in capacity. This is often called the “break-in” period.
- Steady Degradation: After the initial decline, the battery’s capacity degradation tends to follow a more gradual, steady pattern. With each cycle, the capacity gradually decreases. The rate of degradation can vary depending on factors like depth of discharge, temperature, and the specific battery chemistry.
- Accelerated Aging: If the battery experiences extreme conditions, such as high temperatures, deep discharges, or rapid charge/discharge rates, the degradation rate may accelerate. This can result in a more pronounced decline in capacity over a shorter period.
- End of Life: Eventually, the battery will reach a point where its capacity has degraded significantly, and it no longer meets the performance requirements for its intended application. Manufacturers often specify an end-of-life point, often around 80% of the original capacity, at which the battery is considered no longer suitable for its intended purpose.
It’s important to note that not all batteries follow this exact curve, as various factors can influence the shape and steepness of the degradation curve. Different battery chemistries, designs, and usage patterns can lead to variations. Additionally, some modern batteries, particularly those with advanced chemistries like lithium iron phosphate (LiFePO4), may exhibit slower and more predictable degradation curves compared to older technologies.
Battery management systems (BMS) are used to monitor and manage batteries in real-time, helping to prolong their life by optimizing charging and discharging patterns and providing early warnings of potential issues. Regular maintenance and adhering to manufacturer guidelines also play a crucial role in managing battery depreciation and extending their lifespan.
What is the average lifetime of a grid-battery in age or in charging cycles?
The average lifetime of a grid-connected battery can vary significantly depending on several factors, including the battery chemistry, the manufacturer, how the battery is used, and the specific application. However, I can provide a general guideline based on common battery technologies:
- Lithium-Ion Batteries: Lithium-ion batteries are widely used in grid-connected energy storage systems. On average, these batteries are designed to last anywhere from 10 to 20 years in terms of calendar life. This means they can provide useful service for that duration before reaching the end of their expected lifespan.
- Charging Cycles: Lithium-ion batteries are often rated for a certain number of charge-discharge cycles, typically in the range of 1,000 to 5,000 cycles, depending on the specific battery chemistry and design. The depth of discharge and other usage patterns can also affect cycle life. For example, if a lithium-ion battery is regularly discharged to a very low state of charge (deep discharges), it may have fewer cycles before reaching the end of its life.
- Lead-Acid Batteries: Lead-acid batteries are an older technology commonly used in grid applications. They have a shorter lifespan compared to lithium-ion batteries, typically ranging from 5 to 15 years in calendar life.
- Charging Cycles: Lead-acid batteries are often rated for a few hundred to around 1,000 cycles, depending on the specific type (flooded, gel, AGM) and usage conditions. Similar to lithium-ion batteries, the depth of discharge and maintenance practices can influence cycle life.
- Flow Batteries: Flow batteries, which include technologies like vanadium redox flow batteries, offer longer calendar life compared to some other technologies. They are designed to last 15 years or more, with the potential for significantly more cycles.
- Charging Cycles: Flow batteries can offer thousands to tens of thousands of charge-discharge cycles, making them suitable for long-duration energy storage applications.
It’s important to note that these are general estimates, and the actual lifespan of a grid-connected battery will depend on many factors, including how it is used, maintained, and the specific operating conditions. Battery management systems (BMS) and regular maintenance can help maximize the lifespan of grid batteries. Additionally, advancements in battery technology may lead to longer lifespans and improved performance in the future. When evaluating a specific battery system, it’s essential to consult the manufacturer’s specifications and warranties for more accurate information on expected lifespan and performance.
Do have batteries have a residual value at the end of their lifecycle?
Batteries can have residual value at the end of their lifecycle, depending on several factors. The residual value is typically associated with the potential to recycle or repurpose certain components of the battery. Here are some considerations:
- Recycling: Many battery technologies, including lithium-ion batteries and lead-acid batteries, contain valuable materials that can be recycled. Recycling facilities can extract materials like lithium, cobalt, nickel, and lead from spent batteries. These materials can be reused in the production of new batteries or other industrial applications. Recycling can generate revenue and reduce the environmental impact of disposing of batteries in landfills.
- Repurposing: In some cases, even when a battery can no longer meet the performance requirements for its original application, it may still have enough capacity and capability to be repurposed for less demanding tasks. For example, an electric vehicle (EV) battery that no longer meets the range requirements for a car could potentially be used in stationary energy storage applications.
- Second-Life Applications: Some batteries are designed with the idea of a “second life” in mind. After they are no longer suitable for their primary application, they can be used in less critical or lower-performance roles. This can extend the useful life of the battery and provide residual value.
- Core Value: In some cases, there may be a core value associated with the return of used batteries. Manufacturers or recycling facilities may pay a fee for the return of old batteries, especially for large batteries used in applications like electric vehicles.
- Environmental Regulations: Environmental regulations in many regions require responsible disposal and recycling of batteries. These regulations can create incentives for recycling and may affect the residual value of spent batteries.
- Market Demand: The residual value of batteries can also be influenced by market demand for recycled materials and second-life applications. If there is a strong market demand for certain battery components, it can increase their residual value.
It’s worth noting that the residual value of a battery is highly dependent on factors such as the type of battery, its condition, the prevailing market conditions, and recycling infrastructure. As technology advances and recycling processes become more efficient, the residual value of batteries may increase.
In summary, batteries can have residual value through recycling, repurposing, or second-life applications, and their value is influenced by various factors, including market conditions and environmental regulations. Proper disposal and recycling of batteries are not only environmentally responsible but can also have economic benefits.